Nanomaterials for Energy Storage in Lithium-ion Battery Applications
Ganesh Venugopal1, Andrew Hunt1, Faisal Alamgir2
1 nGimat Co., 5315 Peachtree Blvd., Atlanta, GA, USA , 2Georgia Institute of Technology, Department of Materials Science and Engineering 771 Ferst Drive, N.W., Atlanta, GA 30332
Material Matters 2010, 5.2, 42.
Introduction
Rechargeable Lithium-ion (Li-Ion) battery technology has come a long way since its introduction in the early 1990s. Over the last two decades, they have become the technology of choice for powering portable electronic devices such as cellular phones and laptop computers. Currently, Lithium-ion batteries are steadily replacing Nickel-Cadmium (NiCd) and Nickel-Metal Hydride (NiMH) battery technologies in portable power tools. In the future, Lithium-ion batteries are poised to power a new generation of hybrid electric vehicles (HEV), plug-in hybrids (PHEV) and electric vehicles (EV). Another emerging application for Lithium-ion technology is in battery electrical energy storage systems for smart grids that are powered by traditional energy sources like coal, as well as intermittent renewable energy sources like solar and wind.1
The optimum combination of long run time (high energy density at the desired power) and long life (recharge characteristics) sets Lithium-ion battery technology apart from the competition. Needless to say, safety and cost expectations of the application also have to be satisfied. While all of these requirements are being met for portable electronics, the technology is just beginning to move up the optimization curve for emerging applications such as electric automotives, power tools and storage systems. For example, one factor that distinguishes the portable electronics and the electric vehicle application is power density. The latter requires much higher charge and discharge rates compared to the former. While higher power can be achieved to some extent by redesigning the way the battery cell is constructed, nanomaterials are also expected to play a key role in the achievement of high-power capability. Nanomaterial strategies are also being employed to provide better strain accommodation in high-capacity electrodes so that this storage capacity can be extracted reversibly with minimum compromise in cycle life. Alternatively, the stable cycle life and storage life characteristics of “zero-strain” electrode materials can be improved even further by utilizing nanosized versions of these electrodes, thereby providing a new generation of electrical energy storage options for smart grids and back-up power systems.
Overview of Lithium-ion Electrode Materials Chemistries
Conventional Lithium-ion battery materials typically start as 10-50 micron sized particles, which are then coated onto aluminum or copper current collectors along with conductivity enhancers and binders. The work-horse cathode chemistry for the last couple of decades has been Lithium Cobalt Oxide, LiCoO2, (Prod. No., 442704) a layered compound with a distorted rock-salt (α-NaFeO2) structure.2 Some alternative cathodes, like the three dimensional spinel Lithium Manganese Oxide,2 LiMn2O4, (Prod. 725129) have been commercialized only in niche applications, because of performance limitations.2 Carbon-based materials have been the preferred choice for anodes, with some version of graphite being utilized in a majority of the commercially available batteries.3 Battery developers choose electrode materials with the intent to optimize performance from the standpoints of energy, power, cycle life, cost and thermal stability. Until recently, much of this optimization has taken place with the portable electronic application in mind. More recently however, a significant effort is also being spent on new and emerging applications like power tools, electric vehicles and battery electrical energy storage systems. New chemistries have emerged for cathodes including a variety of mixed metal oxides, like LiMn1.5Ni0.5O4 (spinel, Prod. No. 725110 ), LiNi0.33Mn0.33Co0.34O2 (layered) and LiNiCoAlO2 (layered) and metal phosphates, like LiFePO4, LiCoPO4 (Prod. No. 725145 ) and LiMnPO4, (olivine).2,4 For anodes, new materials include oxides such as Lithium Titanates (Li4Ti5O12) & Tin Oxide (SnO2, Prod. No. 549657 ), elemental silicon (Si, Prod. No. 267414) & tin (Sn, Prod. No. 265640 ) and many carbon-based materials.3,5 In many of these new chemistries, having the materials in nanoparticle form or as a nanostructured particle or film is critical to achieving the desired performance dictated by the end application.6
Advantages of Nanomaterials for Lithium-ion Applications
From the battery application perspective, the incentive for implementing a nanomaterial electrode as a Lithium-ion storage material would be to derive significant improvement in energy, power, cycle life or some combination of the same. Nanoparticles or nanopowder electrode materials, i.e., ultrafine versions of the conventional micron-sized electrode powders, are the earliest implementation of nanomaterials science in the Lithium-ion battery application. Indeed, carbon-black, a nanomaterial that has been around for several decades, has been used in Lithium-ion batteries since its early days.7 While carbon-black is used in the electrode, it does not store electrical energy and merely acts as a “passive” conductivity enhancer to improve power capability. However, by designing the “active” energy storage component of the electrode as a nanoparticle, significant performance improvements can be realized for two reasons:
- shorter diffusion lengths for the Lithium-ion travelling from the particle core to the surface where it transfers to the electrolyte and
- higher electrode-electrolyte contact area arising from the inherently high surface areas characteristic of the particles.6
Reducing electrode particle size into the nanoscale regime is also believed to substantially reduce the mechanical stresses caused by volumetric expansion and contraction during charge and discharge. A recently developed model suggests that particles must have a size under a certain critical radius so that the strain produced by intercalation can be accommodated elastically, rather than by plastic deformation, allowing for the full recovery of the original, stable structure.8 Examples of nanomaterials either in nanopowder form or as nanostructured films with wire, rod, whisker or columnar morphologies are actively being pursued to maximize cycle life with minimum compromise in energy density.5,9
Some processing advantages may also be realized while working with nanosized Li-ion storage electrode materials. For example, most Lithium-ion cathode materials are made from precursors containing lithium and other transition metals, which are combined and then heat treated under a variety of conditions to arrive at the desired oxide or phosphate composition. Heat treatment processes can be tedious and energy-intensive, particularly for large particle aggregates where the surface of the aggregate may experience a different thermal profile compared to the bulk. Improper heat treatment leads to nonhomogeneous composition in the material and hence deterioration in performance. While heat treating nanomaterials, it is easier to maintain a homogenous thermal profile throughout the material and thereby produce homogenous compositions without having to resort to energy-intensive processing methods.
Nanomaterials could also enable ultrathin and flexible electrode geometries, which may spawn a new generation of high-rate battery formats for low profile components such as sensors, RFID and flexible devices for consumer and medical applications. Dispersions and inks made using nanopowder electrodes could be used to fabricate low-cost printed batteries via roll-to-roll processing or to integrate thin-film batteries with other devices in printed electronic assemblies made via ink-jet processes.10
Classification and Production of Nanomaterials
The term nanomaterial is typically used to refer to materials that have at least one dimension of less than 100 nm.11 In using the term, there is also an underlying implication that the material has some enhanced property or characteristic compared to larger particle size versions of the same composition. Nanomaterials are not a new class of materials, even though the recent attention that they have been garnering may suggest otherwise. Some of these materials, like volcano dust, have existed in nature forever. Other man-made materials, like carbon black and fumed titania (TiO2), have been around for several decades. One way to classify nanomaterials is to categorize them according to the method by which they are produced, i.e., physical or chemical. The physical method can be further sub-divided into mechanical or phase-change methods. In the physical-mechanical method, particles in the nanometer size range are produced by milling or grinding larger particles of the target composition without any accompanying chemical change.12 This method is commonly referred to as a “top-down” approach to making nanomaterials. In physical-phase change methods, the nanomaterial is created via a phase change process. Examples include direct precipitation, in which a material in solution is precipitated as a solid nanomaterial, and thermal, plasma or laser ablation processing in which vaporized material is condensed into solid nanoparticles. In using either of the physical processes, no chemical change is necessary to arrive at the target nanomaterial composition. Chemical methods include processes where the nanomaterial is synthesized from an initial material that is chemically different from the target composition. The target composition is arrived at via chemical synthesis of a solid that is formed directly in the nanoscale. Examples include flame pyrolysis, spray pyrolysis and wet-chemical methods such as sol-gel and solvo-thermal synthesis.12 The chemical method and the physical-phase change method are both examples of the “bottom-up” approach to making nanomaterials.
An example of a chemical process for making nanomaterials is NanoSpray Combustion, invented by one of the authors, where nanoparticles or nanopowders are produced via combustion chemical vapor condensation (nCCVC).13 In this process, nanoparticles are produced by the combustion of a solution nanospray that consists of precursors that contain the elements that eventually make up the nanoparticle. A schematic of this process is shown in Figure 1. nGimat′s proprietary Nanomiser® device is critical to generating the nanospray from which vapor species are formed in the flame. Condensation of particles can take place either in dry form nanopowders or into a liquid medium to form dispersions. By tailoring precursor formulations and process parameters, nCCVC can be also be used to produce nanomaterials with dopants and surface carbon-coatings to improve performance in the end application.
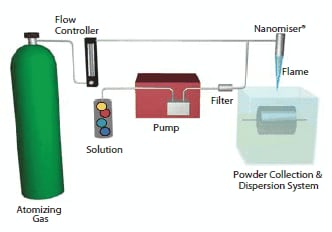
Figure 1.Schematic of the NanoSpray combustion chemical vapor condensation (nCCVC) process for making nanopowders.
While nanoparticles or nanopowders are by definition less than 100 nm in size, other nanomaterials may exhibit structural features that are <100 nm, while existing as larger aggregates like secondary particles or films. For example, lithographic patterning, chemical vapor deposition and physical vapor deposition are all capable of producing nanostructured films with complex structures and are often referred to as form-in-place techniques.12 A variation of nCCVC, called combustion chemical vapor deposition (nCCVD) can also be classified in this category.14 This process has been used to make nanostructured thin film coatings with super-hydrophobic, antimicrobial, oxygen-barrier and moisture-barrier characteristics. The nCCVD process has also been used to deposit battery electrode materials.5 In the following sections, we will focus on the application of nanoparticles or nanopowders in Lithium-ion batteries.
Lithium-ion Battery Application of Nanopowders Made by nCCVC
Using the nCCVC process described above, it is possible to produce a variety of Lithium-ion electrode material compositions that are being pursued by the battery community. The nCCVC process is very wellsuited for making metal oxides and metal phosphate materials. Figure 2 shows the transmission electron micrographs (TEM) of two representative compounds, LiCoPO4 and LiMn1.5Ni0.5O4. The two materials show different particle morphologies; the former shows spherical features, while the latter is composed of faceted particles. In both cases, the average particle size is well under 100 nm. The higher magnification picture of the LiMn1.5Ni0.5O4 nanoparticle reveals the crystalline structure of the nanoparticle.
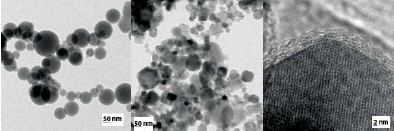
Figure 2.Transmission electron micrographs showing nanoparticles of Lithium Cobalt Phosphate, LiCoPO4 (left), Lithium Manganese Nickel Oxide, LiMn1.5Ni0.5O4 (center), and Lithium Manganese Nickel Oxide, LiMn1.5Ni0.5O4 (right) at higher magnification.
Both LiMn1.5Ni0.5O4 and LiCoPO4 are candidates for high-voltage Li-ion cathodes for a new generation of Lithium-ion batteries.2 For example, LiMn1.5Ni0.5O4 can be charged up to the 4.8–5.0V range compared to 4.2–4.3V charge voltage for LiCoO2 and LiMn2O4.15 The higher voltages, combined with the higher theoretical capacity of around 155 mAh/g for LiMn1.5Ni0.5O4 enable a 25% improvement in energy density compared to conventional Lithium-ion cathodes. Other examples of cathode nanomaterials synthesized by nCCVC include LiCoPO4, spinel LiMn2O4 and orthorhombic LiMnO2 (Prod. No. 725137).
The X-ray diffraction (XRD) patterns of the above-mentioned cathode material and representative anode materials made by nCCVC are shown in Figure 3. All of the patterns match well with reference patterns published by in the literature.16 Candidate anode nanomaterials made by nCCVC include SnO2 and Li4Ti5O12 (Prod. No. 702277). These materials are targeted for different ends of the applications spectrum. SnO2 is a candidate for a high energy density anode with theoretical capacities reaching upward of 750 mAh/g and a voltage of <1V vs. lithium metal, compared to 370 mAh/g and <0.5V for the conventional graphite anodes of today.5,6 Lower voltages are typically desired for anodes so as to maximize the voltage and energy when coupled with a corresponding higher-voltage cathode. Li4Ti5O12, on the other hand, has a capacity of around 160 mAh/g at nominal voltages of around 1.5V.17 Although it has a lower energy density, its zero-strain spinel structure can accept many charge-discharge cycles with a minimum loss in capacity. Furthermore, when used in nanopowder form, Li4Ti5O12 is also known to provide high charge and discharge rate capabilities, as shown below.
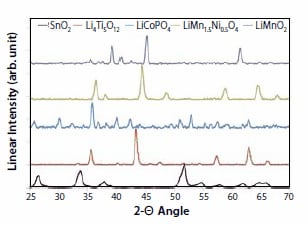
Figure 3.X-ray diffraction patterns for Tin Oxide (SnO2), Lithium Titanate (Li4Ti5O12), Lithium Cobalt Phosphate (LiCoPO4), Lithium Manganese Nickel Oxide (LiMn1.5Ni0.5O4) and Lithium Manganese Oxide (LiMnO2) nanopowders made with the nCCVC process.16
Representative discharge voltage profiles for three of the nanopowder electrodes synthesized by nCCVC are shown in Figure 4 below. This data was acquired with small prototype cells which used Lithium-metal as the counter-electrode. The two curves on the upper end of the chart represent 0.1C-rate profiles for cathode materials LiCoPO4 and LiMn1.5Ni0.5O4 and as anticipated both materials demonstrate highvoltage plateaus in the 4.7–4.8V range. The lower curve represents the C-rate profile for Li4Ti5O12 and this material demonstrates a flat discharge curve with a plateau at around 1.5V.
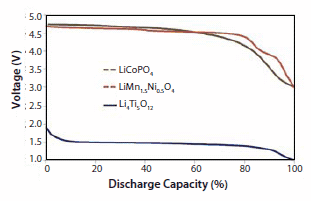
Figure 4.Discharge voltage profiles for three representative Lithium-ion storage nanopowders made by nCCVC and assembled in prototype half-cells with a Lithiummetal anode: Lithium Titanate, Li4Ti5O12 (blue line, bottom) and Lithium Cobalt Phosphate, LiCoPO4 and Lithium Manganese Nickel Oxide, LiMn1.5Ni0.5O4, (red and green lines, top).
The high-rate discharge capability of the nanomaterials has also been demonstrated in prototype cells for the Li4Ti5O12 materials. As shown in Figure 5, Li4Ti5O12 can be discharged at 5C and 10C continuous rates and can retain up to 80% and 60% of the C-rate capacities respectively. In comparison, conventional cathode materials like LiCoO2 are typically not recommended for use over 3C-rates. Typical discharge capacities for the Li4Ti5O12 nanomaterials at C-rate have been around 145 mAh/g. The Li4Ti5O12 materials have also been cycled for >200 cycles at C-rate. This is highly encouraging performance for the nanomaterial, considering that the test-vehicle is only a lab-assembled prototype cell format. The performance of the material can be expected to improve significantly when assembled using processes that are closer to commercial cell manufacturing methods.
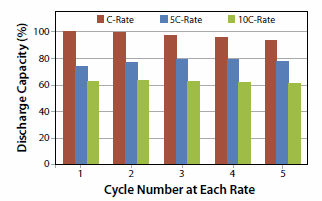
Figure 5.Comparison of the relative capacities achieved at high discharge rates (5C and 10C) vs. capacity achieved at 1C discharge rate over 16 cycles for a Li4Ti5O12-Lithiummetal half-cell in prototype format.
Under the right conditions, the nCCVC process will allow the formation of surface coatings on the electrode particles. Direct coating of the electrode particles with carbon provides higher electronic conductivity to enhance rate capability of the battery. Surface-coated carbon may also eliminate the need for additional conductivity enhancers like carbon-black in the coated electrodes, providing opportunities to increase the energy density of the cell. By tailoring the precursor system and the nCCVC processing conditions, it is possible to make carboncoated Li4Ti5O12 nanopowders with a single step process.12 In conventional methods used to process Lithium-ion electrode materials, a separate down-stream processing step is required for making carboncoated materials.18
Carbon nanotubes (CNT) are also considered to be promising candidates for a high performance Lithium-ion anodes due to their high surface area and also their outstanding electrical and mechanical properties.19 However, the experimental results show only a 20-25% increase for reversible capacity compared to graphite electrode because of weak lithium adsorption to CNTs.20 To improve Li adsorption, researchers have used either chemical/plasma etching for making defects or fullerene (C60) encapsulation of single-walled carbon nanotubes for the creation of fullerene "peapods."21,22 In some cases, materials with a maximum capacity of ~1,000 mAh/g, corresponding to Li2.7C6, are produced. However, these methods require complex and high-temperature processing, often leading to deterioration in their performance.
The Future for Lithium-ion Energy Storage Materials
Emerging applications have steered Lithium-ion materials R&D in a new direction, which includes development of nanomaterial electrodes. Early versions of these nanomaterials are already beginning to appear in limited quantities in the marketplace, primarily in portable power tool applications. Within the next few years, Lithium-ion nanomaterials can also be expected to appear in automotive applications like PHEV and also in battery electrical energy storage systems. Existing applications like portable electronics, where Lithium-ion technology is fully entrenched, could also benefit from the implementation of nanomaterial electrodes that provide high-capacity at the desired powerlevels without compromising cycle life.
While adapting to the electrical performance requirements of the emerging applications, the materials and the processes used to make them have to be aware of the cost-sensitivity in the marketplace. Reduction in raw materials cost and consolidation of multiple steps in the process will be critical to any commercialization effort. Since Lithium-ion electrodes pack more energy and are in the proximity of non-aqueous electrolytes, they will also incur higher packaging costs at the cell-level and at the battery pack-level, compared to Nickel-Metal Hydride systems. Thermal cut-off devices in the cells and safety circuits and thermal management systems in the packs will ensure that the battery is well within its safe operating limit. In the long run, the performance premium offered by Lithium-ion batteries will more than off-set the added costs, particularly with economies of scale.
Acknowledgments
The authors would like to thank other members of the nGimat team for their contribution to the work described in this article. In particular, we would like to acknowledge Michael Sapp, Nina Vylkov, Chris Rockett, Yongdong Jiang and David Krauss. Transmission electron microscopy of our materials was performed by Dr. Yong Ding at the Georgia Institute of Technology. We would also like to acknowledge the financial support provided by U.S. Department of Energy (DOE) through research grants for the development and scale-up of Lithium-ion battery nanomaterials.
References
To continue reading please sign in or create an account.
Don't Have An Account?