Chitosan Biopolymer from Fungal Fermentation for Delivery of Chemotherapeutic Agents
David Brown1, Keith Brunt2, Nils Rehmann3
1CTO Mycodev Group, Fredericton, NB E3B 6B3 Canada, 2Dalhousie School of Medicine New Brunswick, Saint John, NB E2K 5E2, Canada, 3NiRem Consulting, 8 Fish Hatchery Lane, French Village, NB E3E 2H5, Canada
Material Matters, 2016, 11.3
Introduction
Chitosan is a naturally occurring polysaccharide ideally suited for use in medical supplies, devices, therapeutics, and diagnostics. The unique natural characteristics of chitosan include its cationic, biocompatible, biodegradable, non-toxic, non-immunogenic, and antimicrobial properties. Chitosan is predominantly extracted from shellfish waste in developing countries with limited oversight. This causes inconsistencies in molecular weight and purity and allows the introduction of contaminates such as heavy metals in crustacean-derived chitosan. Shellfish allergen protein, such as tropomysin, and high levels of heavy metals, such as mercury and arsenic, may be present in crustacean chitosans, particularly those sourced from regions with high levels of pollution. This lack of product control results in significant risk of reticent anaphylaxis thus, limiting its clinical use. Another production-related issue in the use of chitosan produced from shellfish is the seasonality of the industrial harvest. An alternative approach is the fermentation of fungal chitosan. Pharmaceutical-grade chitosan can be produced from fungi using a submerged fermentation process in a highly controlled and standardized production method using good manufacturing processes (GMP), to produce material acceptable for medical use in drug delivery and other life science applications. Chitosan is a cationic polysaccharide composed of β(1-4) linked glucosamine and N-acetyl glucosamine units1 (Figure 1). Fermentation is a preferred method for production of many clinical compounds due to the ease in which biochemically controlled and standardized engineering conditions can be met, offering an optimal method for the production of chitosan for medical applications.

Figure 1.The glucosamine (1) and N-acetyl glucosamine (2) units of chitosan biopolymer.
Fungal Fermentation for Chitosan Production
Chitin is second only to cellulose as the most abundant biopolymer found in nature. Chitin and its derivative chitosan are naturally produced in fungi as the principal component of the cell walls.2 Chitin and chitosan production in the cell wall is a complex biosynthetic process performed by different families of chitin synthase (CHS) enzymes. A portion of the synthesized chitin is deacetylated to chitosan by the enzyme chitin deacetylase.3 The submerged fermentation of the fungus allows for tightly controlled production of chitosan and limits exposure to foreign material and other organisms.
Mycodev Group, a company producing pharmaceutical-grade fungalderived chitosan, uses a proprietary species of filamentous fungus to produce chitosan using submerged fermentation. While the use of this approach is the first in the chitosan industry, it has been envisioned for some time.
For example, George Roberts of the University of Nottingham mentioned the process in a 2008 review on fungal production stating that this fermentation would offer a stable, non-seasonal source of raw material that is more consistent in character than shellfish waste.4 The aforementioned concerns with shellfish allergen protein and high levels of heavy metals are not applicable to fungal chitosan.
Production of Specific Molecular Weights and Percent Degree of Deacetylation
The most important characteristics in determining the functionality of chitosan are degree of deacetylation (%DDA) and molecular weight (Mw). Chitosan is technically defined as chitin with more than 60% DDA.6 Chitosan with higher %DDA possesses more positively charged amine groups when dissolved in solution.7 In traditionally sourced crustacean based chitosan production, large volumes of high temperature, caustic solution are required to chemically remove the acetyl groups. Fungal production of chitosan allows for very high %DDA values due to the nature of the fermentation, with the ability to routinely produce a DDA as high as 99%.
The average Mw of chitosan is also a very important characteristic. Similar to most polymers, the Mw of chitosan impacts the viscosity of the chitosan solution, whereby increases in the Mw of chitosan raises the viscosity of the solution. Chitosan properties such as biodegradability, mucoadhesion, hemostatic, antimicrobial, anticholesterolemic, and antioxidant ability are all highly dependent on Mw.8 Many applications require covalent or ionic crosslinking of chitosan to other molecules, with the Mw cited as a critical factor in successful chemical conjugation.9 The Mw consistency of chitosan has long been a hurdle to commercial reproducibility and repeatability, significantly hindering the translation of academic studies into commercial applications. Sophisticated applications where chitosan interacts with other substances also suffer from inconsistent results when chitosan with high levels of batch-to-batch Mw variability are used. While crustacean-based production of chitosan exhibits a high degree of batch-to-batch variance in Mw (and difficulty in separating different molecular weights),10 fermentation provides conditions for fungal biomass production with a predictable chitosan Mw. For example, the polydispersity index (PDI) is known to be much lower in fungalsourced chitosan, improving the performance and reproducibility of many chitosan applications. Figure 2 shows a representation of the Mw distribution of fungal chitosan vs. large PDI chitosan from traditional crustacean source methods.
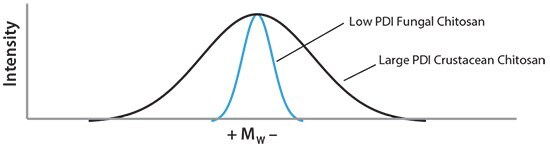
Figure 2.A representation of the polydispersity index (PDI) of chitosan from fungal and crustacean sources. Fungal chitosan typically has a low PDI compared to crustaceansourced chitosan.
The variability of fermentation, such as time, temperature, pH, nutrients, aeration, and mixing, all affect the Mw and PDI of chitosan, yet strict control of these parameters ensures a high degree of reproducibility of the chitosan Mw and %DDA. Consistent Mw and %DDA is critical for the use and acceptance of chitosan in medical and pharmaceutical applications and allows for less variability in research, development, and subsequent commercialization. Fungal chitosan production by fermentation also allows for control of the Mw, allowing the ability to produce a specific Mw to fulfil specific application requirements. Technology developed by Mycodev Group has allowed production of different molecular weights directly as a result of the controlled fermentation process (reproducibly from batch to batch). Through fermentation, molecular weights from as low as 5 kDa to as high as 500 kDa, and any molecular weight in between, can be routinely produced. This ability has become essential for enabling the development of specific chitosan applications and securing paths to commercialization that previously seemed unreachable using the traditional chitosan supply.
Chitosan for Delivery of Chemotherapeutic Agents
Chemotherapy and targeted therapy combinations have helped to significantly improve patient outcomes in the last decade. Though chemotherapeutic agents can be highly effective, systemic administration of these agents has significant adverse side effects on healthy cells, and causes negative physical effects including nausea, hair loss, compromised immune system, cardiotoxicity, and nervous system damage.11
Contained delivery of chemotherapy to cancer-site specific areas reduces or eliminates many detrimental side effects. Additionally, higher concentrations of agents adequately titrated for delivery to a highly specific target area reduce the risk of chemoresistance.12 Polymer and biopolymer drug delivery systems in the form of nanoparticles, films, gels, wafers, and rods have been investigated for their ability to localize chemotherapy administration.13 Biopolymers like chitosan that are biodegradable and can be metabolized from the body are clinically attractive for use in drug delivery. The biocompatibility of chitosan is dependent on the sterility, purity, molecular weight, and percent degree of deacetylation.14
Paclitaxel Encapsulation with ZnO and Modified Chitosan
A recent study using chitosan in combination with ZnO nanocarriers containing the chemotherapy agent paclitaxel (PAC) to target breast cancer tumors gives further insight into the use of chitosan nanoparticles in drug delivery methods. Paclitaxel, a member of the taxane drug class, is limited in the dose that can be provided due to its poor solubility15 and toxicity towards healthy tissues. It was hypothesized that a controlled and localized release of paclitaxel would improve its overall therapeutic efficacy. ZnO as a nanocarrier had still limitations including agglomeration,16 metal oxide toxicity,17 and immune reactivity; however, when chitosan was used as a surface-tethering agent researchers were able to overcome these limitations.
Chitosan-ZnO nanocarriers were conjugated using click chemistry with the alkyne derivative of folic acid for targeted uptake by overexpressed folate receptors in breast cancer tumors (Figure 3). As the chitosan-ZnO nanocarriers arrive in the tumor microenvironment, their surface charge becomes positive, promoting uptake either through surface binding or through folate receptor internalization into the endolysome. Upon delivery, the highly acidic milieu causes the nanocarriers to collapse and release their payload of paclitaxel. As a unique consequence of this collapse, the nanoparticle fluoresces differentially, enabling potential future use in imaging tumor drug titration.

Figure 3.Schematic representation of the approach used to functionalize folic acid through the click chemistry approach for the paclitaxel encapsulated ZnO spheres. Reprinted with permission from Nature Scientific Reports 2015, 5.
In order to test the effectiveness of the chitosan-ZnO nanocarriers, 6–7 week old female athymic BALB/c (nu+/nu+) mice were injected subcutaneously with human MDA-MB-231 adenocarcinoma cells. The paclitaxel-loaded nanocarriers (termed FCPZnO) were tested alongside native paclitaxel, and hollow chitosan-ZnO nanocarriers (HZnO). Tagging IRDye 680 to the FCPZnO prior to the intravenous delivery to the tail vein allowed the researchers to use in vivo fluorescence imaging to track particle uptake to the tumor (Figure 4).
Use of fluorescence imaging made clear that the targeting of the FCPZnO particles to the site of the tumor was significant. The investigators concluded that the preference for folate surface functionalization of the breast cancer cells and the charge repulsion between the healthy tissue and the nanocarriers allowed for the successful delivery of the paclitaxel chemotherapy. Within hours of delivery, FCPZnO was accumulating within the tumor and had a greater than 3-fold efficacy in eliminating tumor volume and mass compared to the control.
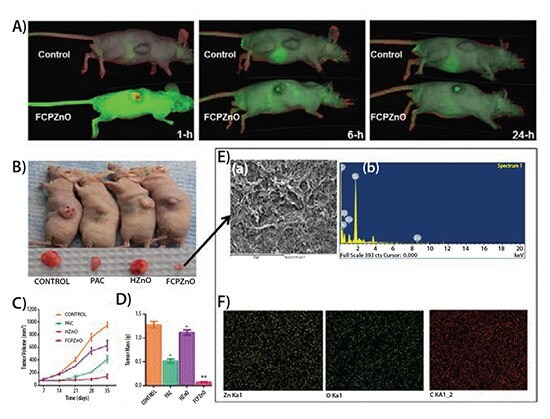
Figure 4.Regression of MDA-MB-231 tumor xenografts in mice treated with FCPZnO and PAC. A) In vivo real-time images of IRDye 680-labeled FCPZnO compared to control (IRDye 680). B) Drug treatment groups received PAC (10 mg/kg, intravenous or iv), equivalent dose of PAC in nanoformulation (10 mg/kg, iv), and equivalent weight of nanocarrier (iv). Tumor volumes in the FCPZnO group were significantly diminished in comparison with free PAC at 35 days after treatment (P <0.05). C) Tumor volume, D) Tumor mass, and E) Spectral shift of tumor sections of FCPZnOtreated mice (A and B). F) FESEM image of tumor cross section area for EDX elemental mapping of corresponding sample zinc (yellow), oxygen (green) and carbon (red) and corresponding EDX spectra. Reprinted with permission from Nature Scientific Reports 2015, 5.
Conclusion
Improving the bioavailability of drugs through novel nanocarrier drug delivery systems is possible through the use of biocompatible substrates like high purity, fungal-derived chitosan. Achieving localized delivery reduces the negative side effects of paclitaxel and proves to be highly effective at eliminating the volume and mass of breast cancer cells in mice. A wide range of drug delivery applications can be enhanced by chitosan prepared either as a hydrogel or as nanoparticles, where the polycationic amino groups interact with the drug’s anionic groups. Chitosan produced by fermentation provides these applications with improved purity and consistency, as well as the opportunity to use specific Mw and %DDA to further optimize the performance and reproducibility.
References
To continue reading please sign in or create an account.
Don't Have An Account?